TARGETED STRATEGIES FOR NANOBOT DRUG DELIVERY
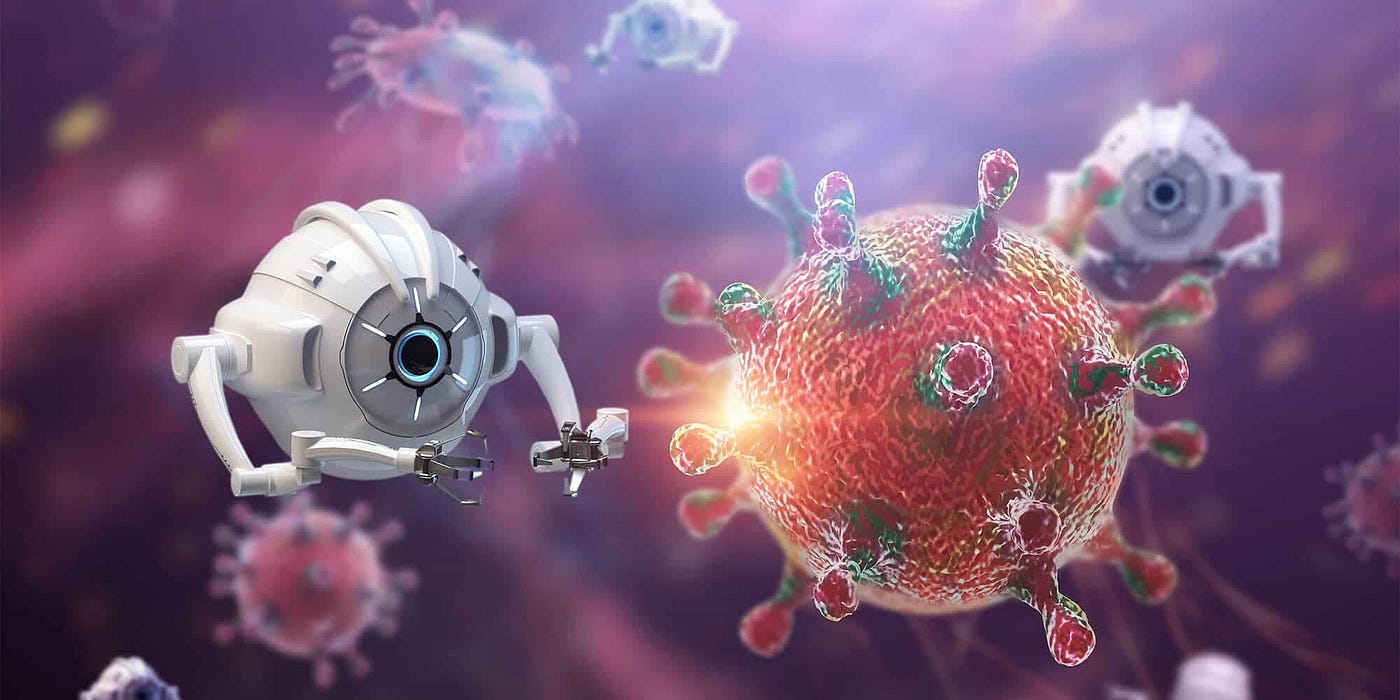
What are drug delivery systems and nanorobots?
Accurate drug delivery has long been a challenge in pharmaceutical research. Ideally, drugs should be delivered directly to target organs, tissues, or cells to maximize therapeutic effects, but traditional methods fall short. Nano carrier-based drug delivery systems offer a promising solution by improving drug solubility, altering distribution, adjusting release rates, and promoting drug aggregation at target sites. Despite extensive research, few nanodrugs enter clinical trials, and even fewer are approved.
Effective drug delivery with nanocarriers often relies on blood circulation for systemic distribution. Tumor tissues, with their unique physiological structures, allow passive targeting nanodrugs to accumulate via the enhanced permeability and retention (EPR) effect. This effect is limited by the body's mechanisms, resulting in minimal nanoparticle accumulation in tumors. Active targeting nanoparticles, designed with ligands that bind to specific cell markers, also face challenges. They cannot autonomously locate targets and depend on blood circulation to reach and bind to receptors in target tissues. Consequently, active targeting's efficiency in tumor accumulation remains suboptimal.
To achieve accurate drug delivery, a system must autonomously propel, navigate, penetrate tissues, tow payloads, and release them precisely. Current nano-carrier systems struggle with these capabilities. Advancements in materials science, molecular biology, mechanics, and artificial intelligence have spurred the development of micro/nanorobots. Proposed by Richard Feynman in 1959, nanorobots are now widely explored for medical applications, including drug delivery.
Unlike traditional methods relying on blood circulation, micro/nanorobots can autonomously move to deliver drugs to hard-to-reach areas. These robots consist of an internal payload and an external shell, powered by external (e.g., magnetic, ultrasound, light) or internal dynamics (e.g., chemical, biological reactions). Micro/nanorobots offer a promising alternative for targeted drug delivery, overcoming the navigation limitations of existing systems.
Micro/nanorobots with autonomous movement ability
Exogenous power-driven micro/nanorobots
Given their micro or nanoscale, drug delivery robots must overcome Brownian motion to navigate through body fluids. Exogenous power sources such as magnetic fields, electric fields, light, sound waves, and heat are often employed to control their movement, sometimes combining multiple methods for enhanced functionality.
Magnetic field propelled micro/nanorobots
Magnetic fields are a popular propulsion method for micro/nanorobots, enabling various swimming strategies like helical swimmers, flexible swimmers, and surface walkers. Inspired by bacterial flagella, helical swimmers use rotating magnetic fields to move, as demonstrated by titanium-coated artificial bacterial flagella (ABF) that achieve precise navigation and drug release under temperature control. The long-term presence of metals in the body poses potential risks, necessitating careful consideration of biocompatibility.
Flexible swimmers, such as the Au–Ag–Ni nanowire, mimic fish movements and are designed for drug delivery. These swimmers can move in response to rotating magnetic fields, with their performance affected by the size ratio between the drug-loaded particles and the nanomotor. Worm-like magnetic nanorobots, with their mesoporous silica nanotube structure, show promise for controlled drug delivery and release in biological fluids.
Surface magnetic walkers and other emerging magnetic field-driven nanorobots also show potential for targeted drug delivery. However, metal components in these robots may cause immune or inflammatory reactions, limiting their in vivo applications.
Electric field propelled micro/nanorobots
Electric fields can drive micro/nanorobots, often in combination with magnetic fields. For instance, a Janus colloidal system uses high-frequency electric fields for movement and cargo delivery, with motion direction controlled by magnetic fields. Electric fields can also regulate catalytic nanomotors' speed and direction. The penetration of electric fields is limited compared to magnetic fields, requiring higher intensities that could damage human tissues.
Light energy propelled micro/nanorobots
Light energy, known for its controllability, is used to drive nanorobots by modulating light frequency, polarization, intensity, and direction. Photocatalytic reactions can also propel nanorobots, as seen with glucose-fueled Cu2O@N-doped carbon nanotube micromotors activated by visible light. Near-infrared (NIR) light, with better tissue penetration and minimal side effects, has shown potential in driving nanorobots.
Ultrasound energy-propelled micro/nanorobots
Ultrasound provides strong propulsion and excellent biocompatibility, making it a promising method for drug delivery. Ultrasound-driven nanorobots, often combined with magnetic fields, can effectively penetrate cells and deliver drugs. However, ultrasound may cause oxidative stress in cells, potentially affecting non-target cells.
Endogenous power-driven micro/nanorobots
Endogenous power sources, such as chemical or biological reactions, also drive nanorobots. These nanorobots often use catalyzers to convert chemical energy from the environment into movement. Hydrogen peroxide decomposition is a common method, but its toxicity limits in vivo applications. Biocompatible alternatives like magnesium and enzyme-catalyzed reactions using non-toxic fuels like glucose and urea are being explored. For example, urease-functionalized nanorobots decompose urea to propel movement and release drugs, demonstrating high efficiency in targeting cancer cells.
Other types of micro/nanorobots
Cell-based micro/nanorobot systems, including red blood cells, bacteria, and stem cells, offer excellent biocompatibility and potential for targeted drug delivery due to their inherent escape mechanisms. These systems often require external stimuli like magnetic fields or sound waves for directional movement. For instance, Shao et al. developed hybrid micromotors using neutrophils and mesoporous silica nanoparticles (MSNs). These micromotors, loaded with E. coli-coated Dox, effectively moved along chemoattractant gradients produced by E. coli. Red blood cells (RBCs) loaded with drugs, quantum dots (QDs), and magnetic nanoparticles (MNPs) demonstrated efficient magnetic guidance and ultrasound-propelled movement, with significantly reduced cytotoxicity compared to drugs alone. Similarly, magneto-aerotactic bacteria like Magnetococcus marinus MC-1, combined with drug-containing nanoliposomes, successfully penetrated tumor hypoxic regions for drug delivery.
DNA origami nanorobots
DNA origami, a technique that uses complementary base pairing to fold single-stranded DNA into specific shapes, is emerging in intelligent drug delivery. These DNA nanostructures can precisely position functional ligands, biomolecules, or nanoscale objects, enhancing targeting capabilities. While DNA origami robots typically lack autonomous movement, they excel in targeted drug delivery. For example, a rectangular DNA origami nanorobot (20 nm × 30 nm) effectively delivered adriamycin to ovarian cancer cells. Other studies have shown DNA origami robots delivering thrombin or ribonuclease A (RNase A) to target cells, demonstrating effective tumor cell killing. Despite these successes, the retention time of these nanorobots in vivo remains a challenge due to immune system clearance.
Application of drug-loaded micro/nanorobots in vivo
The effectiveness of micro/nanorobots for targeted drug delivery has been demonstrated in vitro. Here, we explore their potential in vivo applications and the challenges they face in transitioning from the lab to clinical use. The gastrointestinal tract is a common target for these robots due to its accessibility. For instance, Mg-based microrobots loaded with clarithromycin (CLR) have successfully treated H. pylori infections in mice, showing a significant reduction in bacterial load and confirming their safety. Similarly, Zn-based microrobots have delivered gold nanoparticles to mouse stomachs with high retention rates.
In the intestines, photoacoustic computed tomography (PACT) technology has been used for deep imaging of microrobots. Mg-based micromotors encapsulated with an enteric coating release their payload upon reaching the intestine, driven by a chemical reaction between Mg and water, ensuring gradual drug release. Blood poses more significant challenges due to its complexity. Self-propelled particles using carbonate and tranexamic acid have demonstrated stable movement and effective thrombin delivery for hemostasis in mice and pigs. Other innovative designs include sperm micromotors and multifunctional microrollers for intravascular drug delivery.
Targeting tumors, eyes, and other specific areas has also been explored. For example, Magnetococcus marinus strain MC-1 has delivered drug-loaded liposomes to tumor-hypoxic regions. Additionally, bilayer hydrogel microrobots have been designed for eye treatments, capable of targeted drug delivery and subsequent removal to prevent adverse reactions. In stem cell therapy, 3D porous microrobots driven by magnetic fields offer precise delivery of stem cells for tissue repair. These microrobots have demonstrated the ability to transport cells to specific sites in vivo, such as delivering HeLa cells to mice or human adipose-derived stem cells (hADMSCs) to rabbit knee cartilage lesions.
Table 1. Application of drug-loaded micro/nanorobots in animal models in recent years
Summary and prospect
The field of micro/nanorobots for drug delivery is rapidly evolving, but practical application remains challenging. These robots require precise movement, autonomous delivery, and safe clearance from the body. Advances in materials, 3D printing, and other technologies are expected to enhance their design, bringing us closer to realizing their potential in clinical settings. Future developments may include real-time tracking, in vivo visualization, and bio-inspired designs for improved functionality and safety.
Felfoul, O.; Mohammadi, M.; Taherkhani, S.; De Lanauze, D.; Xu, Y.Z.; Loghin, D.; Essa, S.; Jancik, S.; Houle, D.; LaFleur, M.; et al. Magneto-aerotactic bacteria deliver drug-containing nanoliposomes to tumour hypoxic regions. Nat. Nanotechnol. 2016, 11, 941–947.
Rothemund, P.W.K. Folding DNA to create nanoscale shapes and patterns. Nature 2006, 440, 297–302.
Lu, X.; Liu, J.; Wu, X.; Ding, B. Multifunctional DNA Origami Nanoplatforms for Drug Delivery. Chem. Asian J. 2019, 14, 2193–2202.
Palazzolo, S.; Hadla, M.; Spena, C.R.; Bayda, S.; Kumar, V.; Re, F.L.; Adeel, M.; Caligiuri, I.; Romano, F.; Corona, G.; et al. Proof-of-Concept Multistage Biomimetic Liposomal DNA Origami Nanosystem for the Remote Loading of Doxorubicin. ACS Med. Chem. Lett. 2019, 10, 517–521.
Palazzolo, S.; Hadla, M.; Spena, C.R.; Caligiuri, I.; Rotondo, R.; Adeel, M.; Kumar, V.; Corona, G.; Canzonieri, V.; Toffoli, G.; et al. An Effective Multi-Stage Liposomal DNA Origami Nanosystem for In Vivo Cancer Therapy. Cancers 2019, 11, 1997.
Li, X.; Wang, X.; Li, H.; Shi, X.; Zheng, P. A Programming 20–30nm Rectangular DNA Origami for Loading Doxorubicin to Penetrate Ovarian Cancer Cells. IEEE Trans. NanoBiosci. 2020, 19, 152–157.
Li, S.; Jiang, Q.; Liu, S.; Zhang, Y.; Tian, Y.; Song, C.; Wang, J.; Zou, Y.; Anderson, G.J.; Han, J.-Y.; et al. A DNA nanorobot functions as a cancer therapeutic in response to a molecular trigger in vivo. Nat. Biotechnol. 2018, 36, 258–264.
Zhao, S.; Duan, F.; Liu, S.; Wu, T.; Shang, Y.; Tian, R.; Liu, J.; Wang, Z.-G.; Jiang, Q.; Ding, B. Efficient Intracellular Delivery of RNase A Using DNA Origami Carriers. ACS Appl. Mater. Interfaces 2019, 11, 11112–11118.
De Ávila, B.E.-F.; Angsantikul, P.; Li, J.; Lopez-Ramirez, M.A.; Ramírez-Herrera, D.E.; Thamphiwatana, S.; Chen, C.; Delezuk, J.; Samakapiruk, R.; Ramez, V.; et al. Micromotor-enabled active drug delivery for in vivo treatment of stomach infection. Nat. Commun. 2017, 8, 1–9.
Gao, W.; Dong, R.; Thamphiwatana, S.; Li, J.; Gao, W.; Zhang, L.; Wang, J. Artificial Micromotors in the Mouse’s Stomach: A Step toward in Vivo Use of Synthetic Motors. ACS Nano 2015, 9, 117–123.
Wu, Z.; Li, L.; Yang, Y.; Hu, P.; Li, Y.; Yang, S.-Y.; Wang, L.V.; Gao, W. A microrobotic system guided by photoacoustic computed tomography for targeted navigation in intestines in vivo. Sci. Robot. 2019, 4, eaax0613.
Baylis, J.R.; Yeon, J.H.; Thomson, M.H.; Kazerooni, A.; Wang, X.; John, A.E.S.; Lim, E.B.; Chien, D.; Lee, A.; Zhang, J.Q.; et al. Self-propelled particles that transport cargo through flowing blood and halt hemorrhage. Sci. Adv. 2015, 1, e1500379.
Xu, H.; Medina-Sánchez, M.; Maitz, M.F.; Werner, C.; Schmidt, O.G. Sperm Micromotors for Cargo Delivery through Flowing Blood. ACS Nano 2020, 14, 2982–2993.
Alapan, Y.; Bozuyuk, U.; Erkoc, P.; Karacakol, A.C.; Sitti, M. Multifunctional surface microrollers for targeted cargo delivery in physiological blood flow. Sci. Robot. 2020, 5, eaba5726.
Kim, D.; Lee, H.; Kwon, S.; Sung, Y.J.; Song, W.K.; Park, S. Bilayer Hydrogel Sheet-Type Intraocular Microrobot for Drug Delivery and Magnetic Nanoparticles Retrieval. Adv. Health Mater. 2020, 9, e2000118.
Li, J.; Li, X.; Luo, T.; Wang, R.; Liu, C.; Chen, S.; Li, D.; Yue, J.; Cheng, S.H.; Sun, D. Development of a magnetic microrobot for carrying and delivering targeted cells. Sci. Robot. 2018, 3, eaat8829.
Jeon, S.; Kim, S.; Ha, S.; Lee, S.; Kim, E.; Kim, S.Y.; Park, S.H.; Jeon, J.H.; Kim, S.W.; Moon, C.; et al. Magnetically actuated microrobots as a platform for stem cell transplantation. Sci. Robot. 2019, 4, eaav4317.